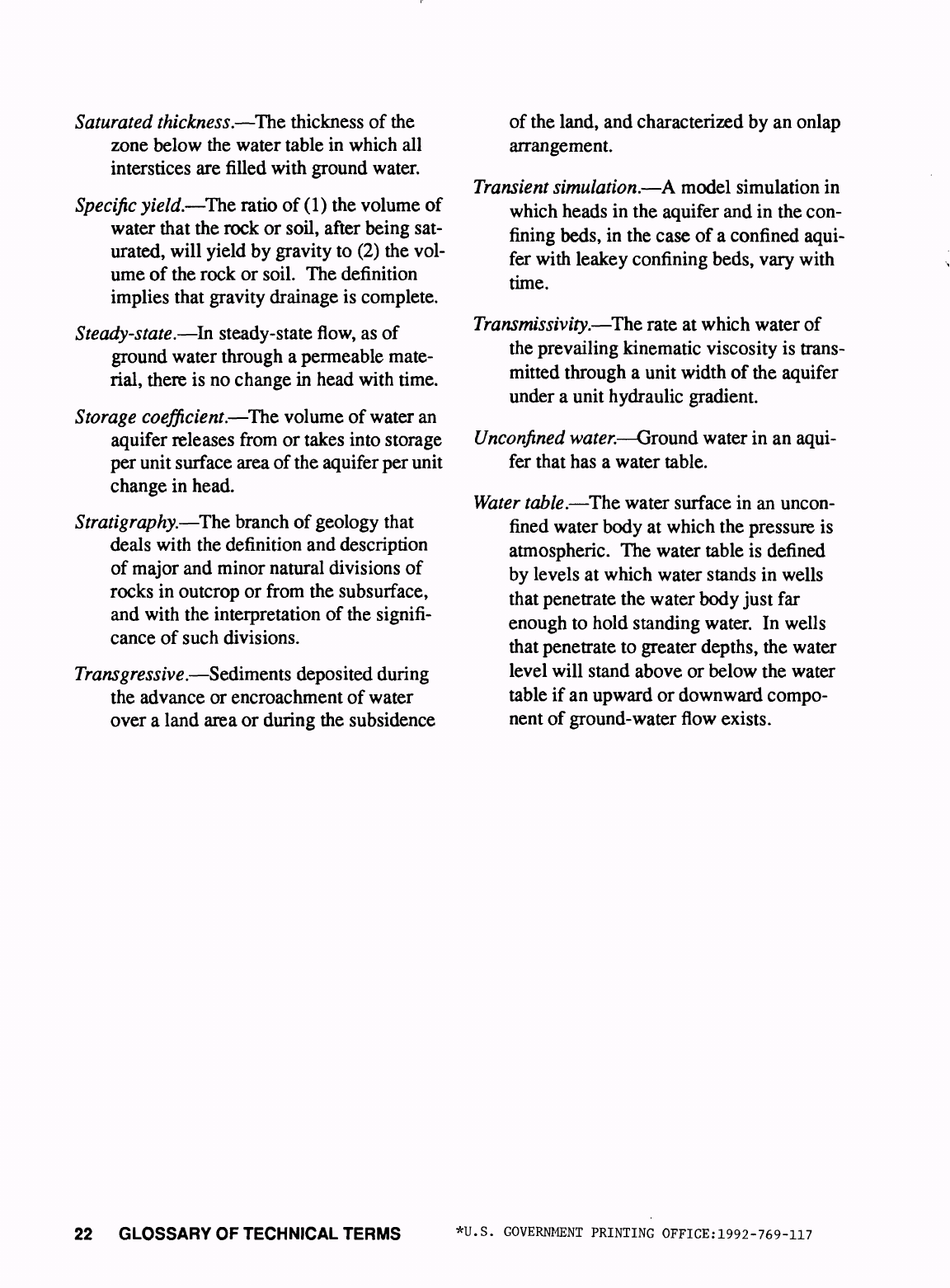
Saturated
thickness.
The thickness
of
the
zone
below
the
water
table
in
which
all
interstices
are
filled
with ground
water.
Specific
yield.
The
ratio
of
(1)
the
volume
of
water
that
the
rock
or
soil,
after
being
sat-
urated,
will yield
by
gravity
to
(2)
the
vol-
ume
of
the
rock
or
soil.
The
definition
implies
that gravity
drainage
is
complete.
Steady-state. In
steady-state
flow,
as
of
ground
water
through
a
permeable
mate-
rial,
there
is
no
change
in
head
with
time.
Storage
coefficient.
The
volume
of
water
an
aquifer releases
from
or
takes
into
storage
per
unit
surface area
of
the
aquifer
per
unit
change
in
head.
Stratigraphy.
The
branch
of
geology
that
deals
with
the
definition
and
description
of
major
and
minor
natural
divisions
of
rocks
in
outcrop
or
from
the
subsurface,
and
with the
interpretation
of
the
signifi-
cance
of
such
divisions.
Transgressive.
Sediments
deposited
during
the
advance
or
encroachment
of
water
over
a
land
area
or
during
the
subsidence
of
the
land,
and
characterized
by
an
onlap
arrangement.
Transient
simulation.
A
model
simulation
in
which
heads
in
the
aquifer
and
in
the
con-
fining
beds,
in
the
case
of
a
confined
aqui-
fer with
leakey
confining beds, vary with
time.
Transmissivity.
The
rate
at
which
water
of
the
prevailing
kinematic viscosity
is
trans-
mitted
through
a
unit
width
of
the
aquifer
under
a
unit
hydraulic
gradient.
Unconfined
water.
Ground
water
in
an
aqui-
fer
that
has
a
water
table.
Water
table.
The
water
surface
in
an
uncon-
fined
water
body
at
which
the
pressure
is
atmospheric.
The
water
table
is
defined
by
levels at
which
water
stands
in
wells
that
penetrate
the
water
body
just
far
enough
to
hold
standing
water.
In wells
that
penetrate
to
greater
depths,
the
water
level
will
stand above
or
below
the
water
table
if
an
upward
or
downward
compo-
nent
of
ground-water
flow
exists.
22
GLOSSARY
OF
TECHNICAL TERMS
*U.S.
GOVERNMENT
PRINTING
OFFICE:1992-769-117